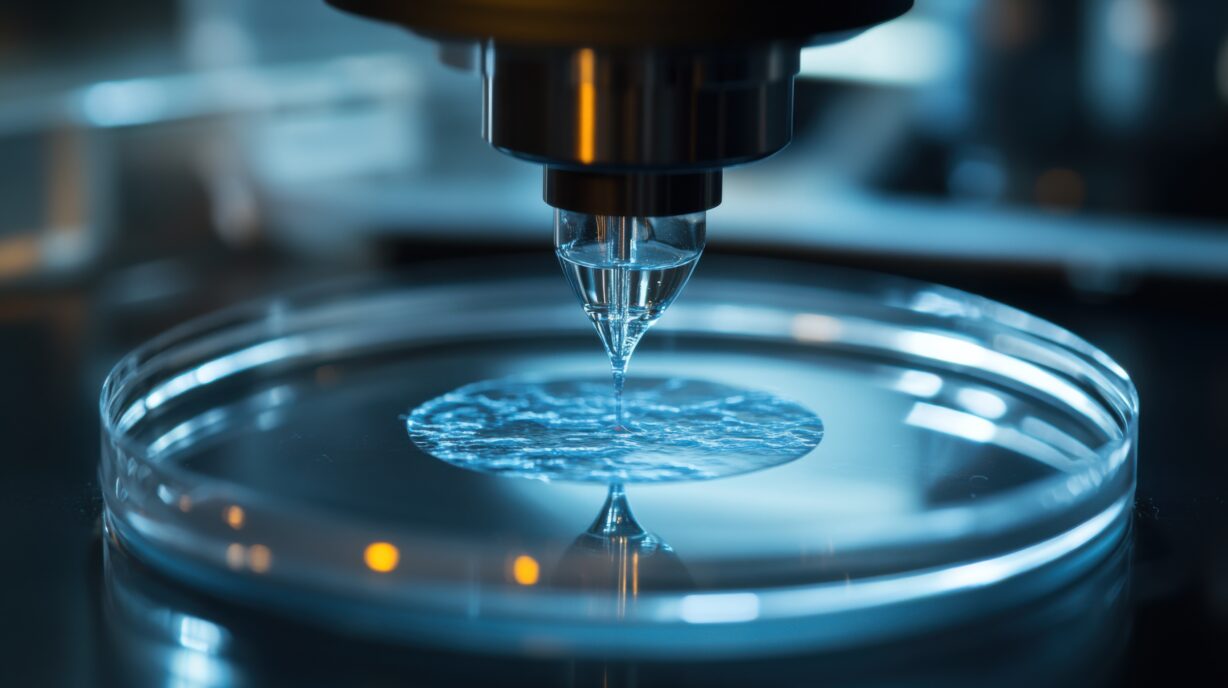
Using Drug Delivery Systems for 3D Bioprinting Tissues
Being able to control the release rate of a target molecule is a valuable tool for engineering tissues and therapeutic delivery of regenerative medicine applications. This blog examines how drug delivery systems can be an important tool for 3D bioprinting tissues using examples from Axolotl Biosciences and the Elvira lab at the University of Victoria.
Many different types of drug delivery systems exist. These systems can be tailored to control the rate and dosage of a target drug being released based on the application. For example, controlled release of cancer therapeutics usually occurs quite rapidly with delivery occurring on the scale of hours while delivering molecules for differentiating stem cells often requires a delivery time course of weeks.
Drug Releasing Microspheres
One exciting new area for applying this technology is the field of 3D bioprinting. 3D bioprinting – a subset of the traditional additive manufacturing technique 3D printing – uses specialized printers to dispense cell laden bioinks into structures that resemble those found in the human body. Both my research group and my start-up biotechnology company – Axolotl Biosciences – have utilized drug delivery systems as an important tool for replicating the conditions found in the in vivo niches that support stem cell differentiation and maturation into functional tissues.
Our team generated a set of drug releasing microspheres fabricated from biocompatible polymer polycaprolactone for neural tissue engineering applications. Microspheres – small spherical particles usually made from biocompatible polymers – can generate controlled release of bioactive factors like small molecules and proteins. We used these drug delivery systems to provide controlled release of factors related to the nervous system with a focus on differentiating stem cells into mature neural tissues.
These microspheres can release retinoic acid – a vitamin A derivative that plays an important role in the development of the neural tube, guggelsterone – a plant steroid that can efficiently differentiate dopaminergic neurons from pluripotent stem cells, and purmorphamine – a Sonic Hedgehog agonist that promotes the formation of motor neurons [1-3]. Since we use these drug releasing microspheres to promote neuronal differentiation, they release drugs over extended time periods of 40 days or longer. Our team incorporated microspheres into hydrogel based bioinks as a way to improve neuronal differentiation when bioprinting neural stem cells.
For example, we used a combination of retinoic acid and purmorphamine releasing microspheres to generate functional spinal cord tissues derived from stem cells as detailed in our Advanced NanoBiomed publication – [4]. Figure 1 shows the results of staining bioprinted spinal cords for mature neural cell markers. These tissues were also electrically active.

More recently, we used our smart bioinks containing purmorphamine releasing microspheres to generate models of Alzheimer’s disease from patient derived stem cells – showing the power of this technology [5]. Figure 2 shows how these bioprinted brain tissues are produced and cultured.
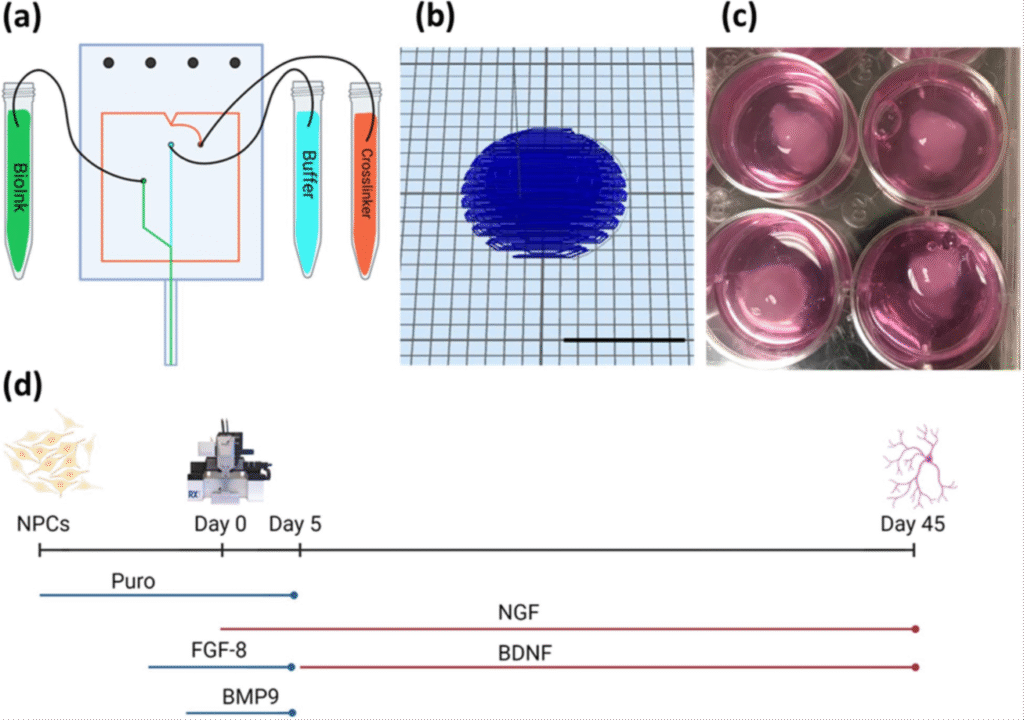
While we initially made these microspheres using a manual emulsion process, this process yielded inconsistent sizes and encapsulation of the target molecules. We began collaborating with Dr. Katherine Elvira’s laboratory to generate more reproducible microspheres using microfluidics. Our collaborative team produced a device for performing this emulsion process that could generate drug releasing microspheres in a more reproducible fashion [6].
Impact of Bioprinting and Microspheres on New Application
Two questions that arise when working with this technology for a new application are how does bioprinting affect the rate of drug release and how do these microspheres affect the mechanical properties of the bioprinted constructs (Figure 4). Our recent publication with the Elvira lab at the University of Victoria led by our former undergraduate research Maria Hangard answered these important questions [7].
Our work demonstrated that suspending the microspheres in bioink did accelerate drug release into the constructs and the microspheres did modulate the mechanical properties of the constructs. Incorporation of the retinoic acid releasing microparticles significantly altered the mechanical properties of the 3D printed fibrin constructs by providing mechanical reinforcement and enhancing the strength and stability of the constructs. The study represents an important step toward developing more sophisticated bioinks that can simultaneously provide appropriate mechanical support and deliver bioactive molecules to guide cell behavior in engineered neural tissues.
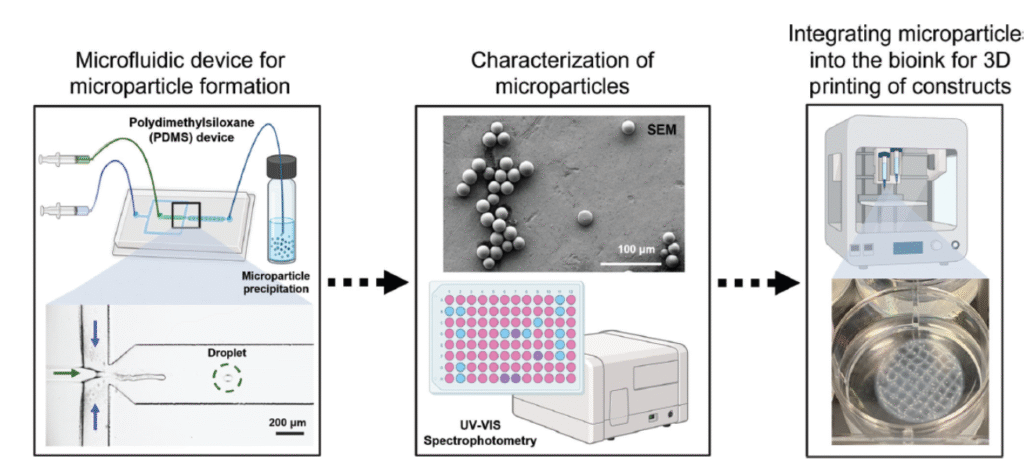
Axolotl Biosciences completed a collaboration with Starfish Medical to commercialize a microfluidic device that would rapidly generate significant quantities of microspheres in a reproducible fashion as detailed by NGen in this case study –https://www.ngen.ca/case-studies/axolotl. This process required the replacement of the polymer based microfluidic device with a larger system made from more durable materials that could be run constantly to produce drug releasing microspheres. Our recent paper published in Advanced Healthcare Materials used these commercial grade microspheres as a tool for engineering a testis model as a way to treat male infertility https://advanced.onlinelibrary.wiley.com/doi/full/10.1002/adhm.202402606 [8] .
Fertility Preservation for Pediatric Cancer Patients
Fertility preservation for pediatric cancer patients has become a major focus in infertility research. Many cancer treatments can damage reproductive tissues, leading to infertility. In vitro spermatogenesis (IVS) can potentially generate functional sperm from stem cells, but recreating the complex architecture and cellular interactions of the testis remains challenging. This study develops a 3D bioprinted coaxial testis model using human induced pluripotent stem cells (hiPSCs) that mimics the bicompartmental cytoarchitecture of the human testis.
The goal was to create a more physiologically relevant model for studying spermatogenesis and potentially advancing fertility preservation techniques. The model recreated the two main compartments of the testis 1) the tubular compartment (seminiferous tubules) where spermatogenesis occurs and 2) the interstitial compartment containing supporting cells and vasculature as shown in Figure 4.
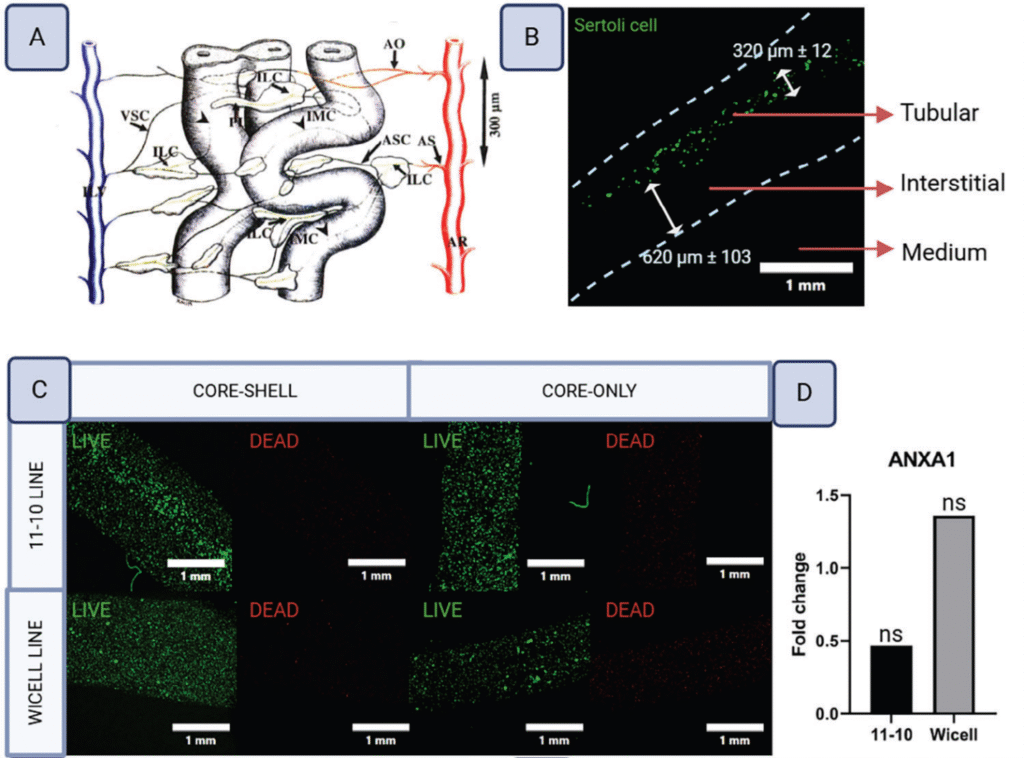
This study also found that incorporation of the commercial grade microspheres into the constructs enhanced the differentiation of this model into functional tissues in comparison to supplementation of retinoic acid in the media – highlighting the importance of using microspheres for delivery.
Conclusion
Overall, this set of studies shows the importance of drug-releasing microspheres as a tool for bioprinting functional tissue models.
Dr. Stephanie Willerth is a biomedical engineer, entrepreneur, and thought leader in 3D bioprinting and neural tissue engineering. As CEO of Axolotl Biosciences and a Full Professor at the University of Victoria, she leads innovations in personalized medicine through bioink development and human tissue models. Her work bridges academia and industry, bringing groundbreaking research into real-world healthcare applications.
References
1. Gomez, J.C., et al., Incorporation of retinoic acid releasing microspheres into pluripotent stem cell aggregates for inducing neuronal differentiation. Cellular and Molecular Bioengineering, 2015. 8(3): p. 307-319.
2. Agbay, A., et al., Guggulsterone-releasing microspheres direct the differentiation of human induced pluripotent stem cells into neural phenotypes. Biomedical Materials, 2018.
3. de La Vega, L., K. Karminin, and S. Willerth, Engineering neural tissue from human pluripotent stem cells using novel small molecule releasing microspheres. . Advanced Biosystems, 2018. In Press.
4. De la Vega, L., et al., 3D Bioprinting Human‐Induced Pluripotent Stem Cells and Drug‐Releasing Microspheres to Produce Responsive Neural Tissues. Advanced NanoBiomed Research, 2021. 1(8): p. 2000077.
5. Benwood, C., et al., 3D bioprinting patient-derived induced pluripotent stem cell models of Alzheimer’s disease using a smart bioink. Bioelectronic Medicine, 2023. 9(1): p. 10.
6. Forigua, A., et al., Microfluidic generation of therapeutically relevant polycaprolactone (PCL) microparticles: computational and experimental approaches. ACS Applied Polymer Materials, 2022. 4(10): p. 7004-7013.
7. Hangad, M.V., et al., Investigating How All‐Trans Retinoic Acid Polycaprolactone (atRA‐PCL) Microparticles Alter the Material Properties of 3D Printed Fibrin Constructs. Macromolecular Bioscience, 2025: p. 2400464.
8. Robinson, M.A., et al., 3D Bioprinted Coaxial Testis Model Using Human Induced Pluripotent Stem Cells: A Step Toward Bicompartmental Cytoarchitecture and Functionalization. Advanced Healthcare Materials, 2025: p. 2402606.
Figure Credits:
Figure 1. Source: Research Article Open Access CC BY 4.0 https://advanced.onlinelibrary.wiley.com/doi/full/10.1002/anbr.202000077 Authors: Laura De la Vega, Laila Abelseth, Ruchi Sharma, Juan Triviño-Paredes, Milena Restan, Stephanie M. Willerth
Figure 2. Source: https://www.frontiersin.org/journals/bioengineering-and-biotechnology/articles/10.3389/fbioe.2020.00057/full Copyright © 2020 Sharma, Smits, De La Vega, Lee and Willerth. This is an open-access article distributed under the terms of the Creative Commons Attribution License (CC BY).
Figure 3. Source: Open Access CC BY-NC-ND 4.0 https://onlinelibrary.wiley.com/doi/full/10.1002/mabi.202400464 Authors: Maria V. Hangad, Alejandro Forigua, Kali Scheck, Stephanie M. Willerth, Katherine S. Elvira
Figure 4. Source: Open Access CC BY 4.0 https://advanced.onlinelibrary.wiley.com/doi/full/10.1002/adhm.202402606 Authors: Meghan A Robinson, Sonia HY Kung, Khaled YM Youssef, Kali M Scheck, Robert H Bell, Funda Sar, Anne M Haegert, M Mahdi Asmae, Changfeng Cheng, Salina V Yeack, Bhairvi T Mathur, Feng Jiang, Colin C Collins, Faraz Hach, Stephanie M Willerth, Ryan K Flannigan