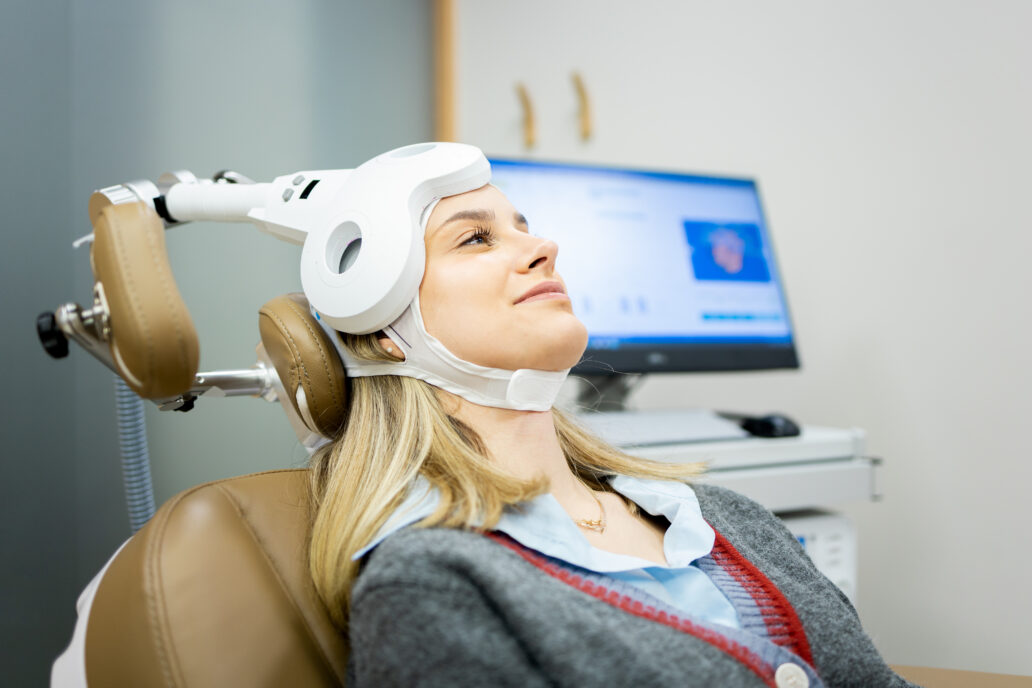
Drug-Device Combination Therapies for Parkinson’s Disease, Epilepsy, Depression and Brain Cancer
Understanding and treating the brain remains one of the greatest challenges in modern medicine. Despite significant advancements, we are still in the early stages of mapping brain regions, deciphering neurological diseases, and optimizing drug delivery to the brain. Addressing these challenges requires innovative approaches that go beyond conventional drug therapies alone. Clinicians are increasingly turning to drug-device combination products, which integrate pharmaceuticals with medical devices to enhance treatment effectiveness.
Obstacles unique to the brain include its intricate neurocircuitry and the protective blood-brain barrier (BBB) which imposes obstacles to traditional intravenous (IV) drug delivery. Devices can help overcome these barriers by improving drug penetration, increasing precision, and reducing systemic side effects. Additionally, some devices can directly modulate neural activity, offering targeted therapeutic effects that drugs alone cannot achieve. As a confounding factor, neurological diseases often involve dysfunction across multiple brain regions, successful interventions must restore neurotransmitter balance, regulate neuronal excitability, and promote neuroplasticity simultaneously.
Combining devices with drugs in a multimodal approach leverages synergistic effects for fine-tuning treatments. These synergistic effects make drug-device combinations a powerful tool in treating neurological disorders that remain difficult to manage with either drugs or devices alone. In this blog, we explore four innovative drug-device combinations that are transforming the treatment of Parkinson’s disease, epilepsy, depression, and brain cancer.
Parkinson’s disease: Combining Levodopa and Deep Brain Stimulation
Parkinson’s disease is a progressive neurodegenerative disorder caused by the loss of dopaminergic neurons in the brain. This leads to motor symptoms such as bradykinesia (slowness of movement), rigidity, tremors, and postural instability (Armstrong & Okun, 2020; Bloem et al., 2021).
The primary treatment for Parkinson’s is Levodopa, a dopamine precursor that replenishes depleted dopamine levels in the brain, helping to alleviate motor symptoms. Carbidopa is often combined with Levodopa to prevent its premature breakdown in the bloodstream, ensuring more of the drug reaches the brain. While Levodopa-Carbidopa therapy is highly effective in early stages, long-term use can lead to motor fluctuations and involuntary movements (dyskinesias), making disease management more challenging (Bloem et al., 2021).
To address these limitations, deep brain stimulation (DBS) can be used alongside Levodopa therapy (Muthuraman et al., 2018). DBS involves implanting a neurostimulator that delivers precise electrical pulses to brain regions like the subthalamic nucleus (STN) or globus pallidus interna (GPi). This neuromodulation helps regulate abnormal brain activity, reducing motor complications and smoothing out fluctuations. DBS can also allow for a lower Levodopa dosage, minimizing its side effects. However, while DBS significantly improves symptoms, it is not a cure on its own and is most effective when combined with pharmacological therapy. Together, Levodopa-Carbidopa and DBS create a powerful synergistic effect, offering patients longer periods of improved mobility with fewer complications (Vizcarra et al., 2019). This multimodal approach highlights the potential of drug-device combination therapies to enhance treatment effectiveness and long-term disease management.
Emerging Technologies to treat Parkinson’s Disease
New technologies aim to refine and enhance the effectiveness of Parkinson’s treatments. One such innovation is adaptive DBS, which differs from conventional DBS by adjusting stimulation levels in real time based on neuronal feedback (Swann et al., 2018). This dynamic approach improves symptom control and reduces side effects compared to fixed-intensity DBS.
Another promising area is gene and cell therapies (CGT), which target the underlying genetic and cellular defects in Parkinson’s disease. These advanced biological therapies often require precise delivery to specific brain regions, necessitating the development of customized, image-guided drug delivery systems.
The integration of DBS and Levodopa-Carbidopa infusion represents a powerful therapeutic strategy for advanced Parkinson’s disease, leveraging both neuromodulation and pharmacotherapy to optimize patient outcomes. Future advancements in closed-loop adaptive DBS and image-guided surgical drug delivery for CGT will further personalize treatment, offering greater precision, improved symptom control, and better long-term disease management for Parkinson’s patients.
Epilepsy: Combining AEDs with neurostimulators
Epilepsy is a chronic neurological disorder characterized by recurrent seizures, which result from abnormal electrical activity in the brain. Depending on the type and severity of epilepsy, seizures can range from mild sensory disturbances to severe convulsions and loss of consciousness (Manole et al., 2023).
The primary treatment for epilepsy involves antiepileptic drugs (AEDs), which help stabilize neuronal activity and prevent excessive electrical discharges in the brain. Common AEDs include levetiracetam, valproate, lamotrigine, and carbamazepine, each targeting different mechanisms to reduce seizure frequency and severity (Kanner & Bicchi, 2022). While AEDs are effective for many patients, a significant portion—often referred to as drug-resistant epilepsy—fails to achieve adequate seizure control with medication alone.
To address this, neuromodulation devices such as vagus nerve stimulators (VNS) and responsive neurostimulators (RNS) are used in combination with AEDs (Wang et al., 2020). VNS therapy involves implanting a small device in the chest that sends regular electrical pulses to the vagus nerve in the patient’s neck, helping to regulate brain activity and reduce seizure frequency. RNS therapy, on the other hand, continuously monitors brain activity and delivers targeted electrical stimulation directly to the brain to prevent seizures before they start. These approaches can significantly reduce seizure burden in patients who do not respond to medication alone.
Emerging Technologies to treat drug-resistant epilepsy.
Advancements in epilepsy treatment are focusing on closed-loop neuromodulation, where devices dynamically adjust precision and reduce unnecessary stimulation, leading to fewer side effects and better long-term seizure control (Anderson et al., 2023). Additionally, new AEDs in clinical trials show promise with fewer side effects and improved pharmacokinetic profiles, including precision medicine approaches that tailor treatments based on genetic and metabolic factors (Sánchez et al., 2024).
While challenges remain, ongoing advancements in adaptive neurostimulation and pharmacotherapy are expected to further improve outcomes for epilepsy patients. As technology evolves, personalized, multimodal treatment approaches will play a central role in optimizing seizure control for those with drug-resistant epilepsy.
Depression: Drug-Device Combinations for Treatment-Resistant Depression
Depression is a widespread and debilitating mental health disorder characterized by persistent sadness, loss of interest, fatigue, and cognitive impairments (Richards, 2011). While conventional antidepressants, such as selective serotonin reuptake inhibitors (SSRIs) and serotonin-norepinephrine reuptake inhibitors (SNRIs), are effective for many patients, treatment-resistant depression (TRD) remains a significant challenge (Moncrieff et al., 2023).
For patients who do not respond to medication alone, brain stimulation therapies offer a promising alternative. Transcranial magnetic stimulation (TMS) and deep brain stimulation (DBS) have emerged as valuable neuromodulation techniques (D’Onofrio et al., 2023). TMS is a non-invasive therapy that delivers magnetic pulses via a device located against the skull to stimulate underactive brain regions, such as the dorsolateral prefrontal cortex, which is associated with mood regulation. DBS, typically used for severe cases, involves implanting electrodes into deep brain structures like the subgenual cingulate cortex to modulate neural circuits involved in depression.
Emerging Technologies to treat Depression
Innovations in depression treatment include personalized, AI-driven brain stimulation systems that adapt therapy in real time based on individual brain activity. Closed-loop DBS, which continuously adjusts stimulation to match a patient’s changing emotional and cognitive states, is being explored to further refine neuromodulation therapy (Scangos et al., 2021).
Additionally, ketamine and psychedelic-assisted therapies, such as those using psilocybin or 3,4- Methylenedioxymethamphetamine (MDMA), have shown promise in rapidly alleviating depression symptoms. These novel pharmacological approaches may be enhanced by neuromodulation techniques, creating a new frontier in depression treatment (Dahan et al., 2024).
By combining pharmacotherapy with neuromodulation, drug-device approaches expand treatment options for patients with severe or drug-resistant depression, improving response rates and long-term mental health outcomes.
Brain Cancer: Revolutionizing Treatment with Drug-Device Integration
Brain cancer, particularly glioblastoma multiforme (GBM), is one of the most aggressive and treatment-resistant malignancies (Wu et al., 2021). Traditional approaches—surgery, chemotherapy, and radiation therapy—have limited success due to the tumor’s infiltrative nature and the challenge of delivering drugs across the blood-brain barrier (BBB).
To enhance treatment efficacy, tumor-treating fields (TTFields) and localized drug delivery systems are being integrated into the standard of care. TTFields therapy involves wearable devices that generate low-intensity electric fields to disrupt cancer cell division, slowing tumor progression (Guo et al., 2022). When used alongside standard chemotherapy drugs for GBM (e.g. temozolomide), TTFields have been shown to improve survival rates.
Another technology that has been used successfully to bypass the BBB is focused ultrasound (FUS) combined with microbubbles. FUS has emerged as a non-invasive method to temporarily and reversibly disrupt the BBB, enhancing chemotherapy penetration into the tumor microenvironment (Hersh et al., 2022). This drug-device combination therapy offers a novel approach that increases chemotherapy efficacy, by increasing drug penetration into the brain.
Emerging technologies to treat brain cancer
New developments include implantable drug-delivery wafers, which release chemotherapy drugs directly into the tumor site after surgical resection, bypassing the BBB and increasing local drug concentration (Ashby et al., 2016). Additionally, nanotechnology-based drug carriers are being explored to deliver chemotherapy more precisely while reducing systemic toxicity (Qindeel et al., 2024).
The integration of chemotherapy, TTFields, FUS and localized drug delivery systems represents a multimodal approach to prolonging survival and improving quality of life for patients with brain cancer. Future advancements in nanomedicine and bioengineered drug delivery systems will continue to refine and personalize treatment strategies.
Figure 1. Schematic of different brain treatment devices and methodologies.
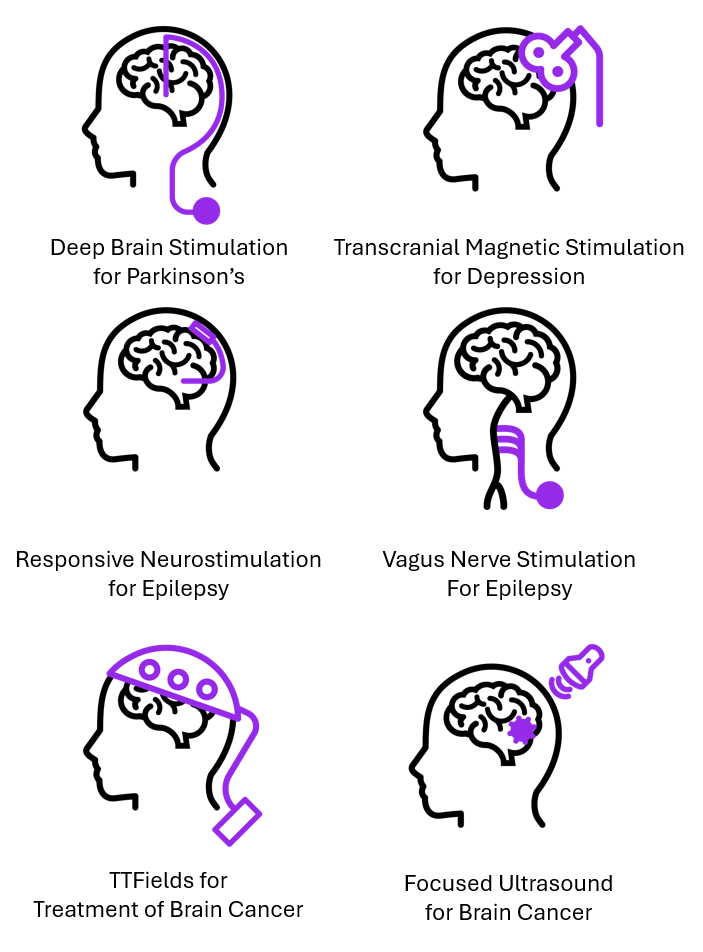
Conclusion
The treatment of brain diseases remains one of the most complex challenges in modern medicine, requiring multimodal approaches that extend beyond traditional pharmacological therapies. Whether through targeted drug delivery systems that bypass the blood-brain barrier or by combining drugs with neuromodulation for conditions like Parkinson’s, epilepsy, and depression, drug-device combination therapies provide greater therapeutic benefits than either approach in isolation. In Fig. 1, you can find an overview of the different brain treatment devices that were discussed in this blog. As these technologies continue to evolve, brain disease treatment will become increasingly personalized, adaptive, and effective. This progress will not only enhance patients’ quality of life but also redefine the future of neurological care, making previously untreatable brain diseases more manageable—and, ultimately, more treatable.
Joris van der Heijden is Concept Development Lead Bio Services at StarFish Medical. Previously he was Director of R&D at Spartan Bioscience where he led the development of a point-of-care COVID-19 diagnostic test. Joris received his PhD in infectious diseases from UBC.
Images: Adobe Stock
References
Anderson, D. N., Charlebois, C. M., Smith, E. H., Davis, T. S., Peters, A. Y., Newman, B. J., Arain, A. M., Wilcox, K. S., Butson, C. R., & Rolston, J. D. (2023). Closed-loop stimulation in periods with less epileptiform activity drives improved epilepsy outcomes. Brain, 147(2), 521–531. https://doi.org/10.1093/brain/awad343
Armstrong, M. J., & Okun, M. S. (2020). Diagnosis and Treatment of Parkinson Disease: A Review. JAMA, 323(6), 548–560. https://doi.org/10.1001/jama.2019.22360
Ashby, L. S., Smith, K. A., & Stea, B. (2016). Gliadel wafer implantation combined with standard radiotherapy and concurrent followed by adjuvant temozolomide for treatment of newly diagnosed high-grade glioma: a systematic literature review. World Journal of Surgical Oncology, 14(1), 225. https://doi.org/10.1186/s12957-016-0975-5
Bloem, B. R., Okun, M. S., & Klein, C. (2021). Parkinson’s disease. The Lancet, 397(10291), 2284–2303. https://doi.org/10.1016/S0140-6736(21)00218-X
D’Onofrio, V., Manzo, N., Guerra, A., Landi, A., Baro, V., Määttä, S., Weis, L., Porcaro, C., Corbetta, M., Antonini, A., & Ferreri, F. (2023). Combining Transcranial Magnetic Stimulation and Deep Brain Stimulation: Current Knowledge, Relevance and Future Perspectives. Brain Sciences, 13(2). https://doi.org/10.3390/brainsci13020349
Dahan, J. D. C., Dadiomov, D., Bostoen, T., & Dahan, A. (2024). Meta-correlation of the effect of ketamine and psilocybin induced subjective effects on therapeutic outcome. Npj Mental Health Research, 3(1), 45. https://doi.org/10.1038/s44184-024-00091-w
Guo, X., Yang, X., Wu, J., Yang, H., Li, Y., Li, J., Liu, Q., Wu, C., Xing, H., Liu, P., Wang, Y., Hu, C., & Ma, W. (2022). Tumor-Treating Fields in Glioblastomas: Past, Present, and Future. Cancers, 14(15). https://doi.org/10.3390/cancers14153669
Hersh, A. M., Bhimreddy, M., Weber-Levine, C., Jiang, K., Alomari, S., Theodore, N., Manbachi, A., & Tyler, B. M. (2022). Applications of Focused Ultrasound for the Treatment of Glioblastoma: A New Frontier. Cancers, 14(19). https://doi.org/10.3390/cancers14194920
Kanner, A. M., & Bicchi, M. M. (2022). Antiseizure Medications for Adults With Epilepsy: A Review. JAMA, 327(13), 1269–1281. https://doi.org/10.1001/jama.2022.3880
Manole, A. M., Sirbu, C. A., Mititelu, M. R., Vasiliu, O., Lorusso, L., Sirbu, O. M., & Ionita Radu, F. (2023). State of the Art and Challenges in Epilepsy-A Narrative Review. Journal of Personalized Medicine, 13(4). https://doi.org/10.3390/jpm13040623
Moncrieff, J., Cooper, R. E., Stockmann, T., Amendola, S., Hengartner, M. P., & Horowitz, M. A. (2023). The serotonin theory of depression: a systematic umbrella review of the evidence. Molecular Psychiatry, 28(8), 3243–3256. https://doi.org/10.1038/s41380-022-01661-0
Muthuraman, M., Koirala, N., Ciolac, D., Pintea, B., Glaser, M., Groppa, S., Tamás, G., & Groppa, S. (2018). Deep Brain Stimulation and L-DOPA Therapy: Concepts of Action and Clinical Applications in Parkinson’s Disease. Frontiers in Neurology, 9, 711. https://doi.org/10.3389/fneur.2018.00711
Qindeel, M., Irfan, M., Ullah, S., Fathi-karkan, S., Kharaba, Z., Rahdar, A., Aliahmad, M., & Aboudzadeh, M. A. (2024). Nanotechnology in glioblastoma therapy: Advances in drug delivery systems and diagnostic approaches. Journal of Drug Delivery Science and Technology, 102, 106322. https://doi.org/https://doi.org/10.1016/j.jddst.2024.106322
Richards, D. (2011). Prevalence and clinical course of depression: A review. Clinical Psychology Review, 31(7), 1117–1125. https://doi.org/https://doi.org/10.1016/j.cpr.2011.07.004
Sánchez, J. D., Gómez-Carpintero, J., González, J. F., & Menéndez, J. C. (2024). Twenty-first century antiepileptic drugs. An overview of their targets and synthetic approaches. European Journal of Medicinal Chemistry, 272, 116476. https://doi.org/https://doi.org/10.1016/j.ejmech.2024.116476
Scangos, K. W., Khambhati, A. N., Daly, P. M., Makhoul, G. S., Sugrue, L. P., Zamanian, H., Liu, T. X., Rao, V. R., Sellers, K. K., Dawes, H. E., Starr, P. A., Krystal, A. D., & Chang, E. F. (2021). Closed-loop neuromodulation in an individual with treatment-resistant depression. Nature Medicine, 27(10), 1696–1700. https://doi.org/10.1038/s41591-021-01480-w
Swann, N. C., de Hemptinne, C., Thompson, M. C., Miocinovic, S., Miller, A. M., Gilron, R., Ostrem, J. L., Chizeck, H. J., & Starr, P. A. (2018). Adaptive deep brain stimulation for Parkinson’s disease using motor cortex sensing. Journal of Neural Engineering, 15(4), 46006. https://doi.org/10.1088/1741-2552/aabc9b
Vizcarra, J. A., Situ-Kcomt, M., Artusi, C. A., Duker, A. P., Lopiano, L., Okun, M. S., Espay, A. J., & Merola, A. (2019). Subthalamic deep brain stimulation and levodopa in Parkinson’s disease: a meta-analysis of combined effects. Journal of Neurology, 266(2), 289–297. https://doi.org/10.1007/s00415-018-8936-2
Wang, A. J., Bick, S. K., & Williams, Z. M. (2020). Vagus Nerve Stimulation versus Responsive Neurostimulator System in Patients with Temporal Lobe Epilepsy. Stereotactic and Functional Neurosurgery, 98(1), 21–29. https://doi.org/10.1159/000504859 Wu, W., Klockow, J. L., Zhang, M., Lafortune, F., Chang, E., Jin, L., Wu, Y., & Daldrup-Link, H. E. (2021). Glioblastoma multiforme (GBM): An overview of current therapies and mechanisms of resistance. Pharmacological Research, 171, 105780. https://doi.org/https://doi.org/10.1016/j.phrs.2021.105780