Optical Technologies for Point of Care
An overview of optical technologies for point of care (POC) medical devices that have been developed or show promise. Point-of-care (POC) testing is diagnostic testing conducted at or near the location where a patient is receiving medical care (away from a clinical laboratory).
POC diagnostic devices allow for rapid diagnoses immediately following the collection of samples from patients. This contrasts with how medical testing has historically been done, in which samples are sent away for testing and results are received several days later.
Rapid testing can improve outcomes in cases where time is a factor. POC testing can also streamline the testing process, as fewer people and less labour can be required to reach a diagnosis. Finally, POC testing devices have the power to improve access to health care by bringing testing to the patient instead of the other way around. The medical impact on patients who have difficulty coming to a clinic, or who live in remote communities, could be immense.
Today, POC devices have been developed for a wide and expanding range of diagnostic tests [1, 2]. A variety of techniques are used to enable the detection of analytes (i.e. substances or chemicals of interest) in POC devices. They include lateral flow assays, electrochemical sensor methods, and optical detection methods.
Some POC tests, such as pregnancy test kits and blood glucose monitors (which generally use lateral flow and electrochemical methods, respectively), are already commonplace and can be purchased over-the-counter.
POC devices are designed to be affordable, accurate, user-friendly, robust, rapid, and portable. The World Health Organization has summarized these requirements in providing guidance for features that should be designed into POC tests for the detection of sexually transmitted infections (STIs) through the acronym ASSURED (Affordable, Sensitive, Specific, User-friendly, Rapid and Robust, Equipment-free – no complex equipment, Delivered). It can be challenging to simultaneously meet all these requirements and some compromises may be acceptable [3].
Optical detection schemes are generally preferred to other methods because of their high-sensitivity, quantitative accuracy, and capacity for multiplexing (measuring multiple signals/analytes with a single device) [4]. However, miniaturizing optical components without unacceptably sacrificing performance and while reducing cost is a significant challenge. The sections below cover optical technologies that are making their way into POC diagnostic devices.
Miniaturized Microscope Optical technologies for point of care
Microscopes are an invaluable tool in medical diagnosis. Microscopic examination of patient samples remains the gold standard for diagnosing various diseases [1]. The most familiar type of microscopy is bright-field microscopy, in which the sample is illuminated by white light and inspected visually at high magnification.
Also of particular importance is fluorescence microscopy, in which the microscope incorporates a light source and optical filters used to excite and capture emitted light from fluorescent samples. This method is commonly used with samples where a fluorescent tag is made to selectively bind with a known analyte for detection. Inexpensive, small, consumer-grade bright-field-like microscopes can be purchased from many sources, whereas miniature fluorescence microscopes are coming on the market, but are less widely available.
Many fluorescence microscopes are bulky and expensive. Significant miniaturization and cost reduction is required to make them practical for point of care devices. Even using mostly conventional optics, fluorescence microscopes can be made to be inexpensive, battery-powered, and small enough to be considered portable.
One such microscope, the so-called “Global Focus microscope” [5], is only 7.5 cm x 13 cm x 18 cm in size, has a mass of 1 kg, and is estimated by the authors to have a manufactured cost of $240 USD. The microscope can do both bright-field and fluorescence microscopy, with a battery-powered flashlight being used as the illumination and excitation light source. Its ability to detect a human pathogen (specifically, the mycobacterium tuberculosis), showed comparable performance to a conventional Nikon microscope.
It is possible to make microscopes much smaller even than that described above. A video fluorescence microscope has been demonstrated that is only 0.84 cm x 1.3 cm x 2.2 cm in size, with a mass of only 1.9 g [6]. Surprisingly, even in this very small form-factor the optical components used were largely off-the-shelf from large optics suppliers. These components were small but not dissimilar in principle to those used in conventional microscopes.
Read more on medical device miniaturization options and trade-offs.
Notably, a gradient-index (GRIN) lens (an optic that uses a gradient in the refractive index within its volume to affect the beam path) was used as the objective. Such objectives have also been used in endoscopes. While the authors didn’t estimate a final cost, the microscope was designed with cost in mind; all the components chosen were mass-producible, and therefore the cost was expected to be low at volume.
In particular, the optoelectronic components (the camera sensor and the LED used for illumination) were estimated to cost $1-10 USD at volume. This microscope was developed for live animal studies, but its potential for use in POC applications has been noted [1, 6].
Microscopic images must be interpreted for diagnosis and it may not always be practical to have a pathologist on-site at a point-of-care. Provided an internet connection is available, sample images can be sent for diagnosis via properly secured channels to a pathologist (or a team of pathologists) working remotely. It is also likely that AI-based diagnoses, which could be performed using a sufficiently-powerful local computer or via cloud-services, will become more common in the coming decade [7].
Lens-Free Imaging optical technologies for point of care
Perhaps the most effective way to reduce the cost and size of optics is to remove them entirely. A number of optical imaging methods have been developed that remove lenses from the optical path, and instead use the signals recorded by a bare image sensor (such as a CMOS array) along with novel illumination and computation schemes to produce high-quality microscopic images.
Two such techniques are lensless contact imaging and lensless holographic imaging. These methods have advanced in recent years as image sensors have become smaller, cheaper, and higher resolution, and computer processing power has increased.
In lensless contact imaging, the sample is placed directly on top of an image sensor separated only by a very thin transparent layer used to protect the sensor. The sample is illuminated from the back and the resultant shadow is taken as an image of the object [1].
It is important that the sample be very close to the sensor to reduce artifacts from light diffracted around the edges of the object (which would manifest as light and dark fringes around the edge of the object’s shadow). For this reason, it is necessary to eliminate any additional optics from the surface of the sensor, such as optical filters or protective glass windows, which are standard on many image sensors.
Lensless holographic imaging uses the diffraction effects that are suppressed in lensless contact imaging. In this method, the sample is placed a small distance away (1-5 mm) from a bare image sensor. It is illuminated from behind by a coherent light source (such as a quasi-monochromatic LED shone through a pinhole).
This arrangement generates diffraction patterns of the object being imaged on the sensor. Using mathematical methods that are beyond the scope of this blog, an image of the sample can be generated based on the measured diffraction pattern.
Although the pixels on modern image sensors can be very small (~0.8 μm in the most extreme case at present [8]), they still limit the achievable resolution of the microscopic images produced, which are often intended to image features on the nanoscale.
To get around this limit, computational “superresolution” methods are sometimes used [9, 10]. In one implementation, the position and/or angle of the light source is slightly shifted while capturing images at each new orientation. The shifting slightly changes how the light is registered on the detector. By using feature-based image alignment and applying mathematical optimization methods, sub-pixel information can be inferred, leading to higher resolution images than would be possible to record directly from the image sensor.
Smartphone-Based optical technologies for point of care
Smartphones commonly encompass a multitude of sensors and functionalities. The primary optical components (the screen, the flashlight, and the camera) have been applied in developing devices that are amenable to POC applications.
Smartphone touchscreens are used in several different ways. The most straightforward relevant application is to simply use the touch screens as intended: to display the UI and other information to the user and to allow them to interact. While this may seem trivial, it may (in conjunction with the use of the smartphone’s processing power) eliminate the need for any additional external computer or display.
A more novel application is to use the screen as an illumination source. In the particular implementation of lensless contact imaging described above [9], the light source was a phone screen and the light source shifting was done simply by changing which part of the screen was illuminated. The screens have also been used with a specialized eyepiece to display patterns that enable a user to self-evaluate cataract severity [11].
The cameras on smartphones are of particular utility. These cameras have become increasingly compact and high-quality and are able to produce photographs on par with many dedicated cameras. Numerous smartphone-based microscope attachments have been demonstrated with intended POC applications, some also use the flashlight as the illumination source (similar attachments are also available on the consumer market) [12].
Several fluorescence microscope attachments have also been developed for smartphones [13]. One implementation of a cell-phone based fluorescence microscope also incorporated a microfluidic channel in front of the camera, and was used to capture video used for flow cytometry (cell counting in this case) [14]. The video was processed on a separate PC, though the authors noted it could be implemented directly on the smartphone.
Microfluidics Platforms and Optical technologies for point of care
Microfluidics is a key technology for POC diagnostic devices. Generally, microfluidic devices use microscale (< 1 mm) fluid channels to allow small volumes of patient samples to be processed (separated, transported, temperature-controlled, mixed with reagents, etc.) and their properties measured.
Such devices have huge potential for POC devices because of their small size and their capacity for automation, high-sample counts, and multiplexing. Many of the features needed to process the samples (channels, reservoirs, inlets, etc.) are generally manufactured into inexpensive microfluidic chips or cartridges.
Detection can be done in various ways, with optical detection being among the most common. Optical microfluidic detection platforms can measure a sample’s absorption, fluorescence, and chemiluminescence.
Optical interferometric methods, methods based on surface plasmon resonance (used to measure sample adsorption on a functionalized surface), and various imaging modalities are also possible [15].
Conventional, bench-top detection platforms can be used with microfluidic cartridges in lab settings. For POC applications, however, portable detection platforms are preferred. Indeed, many portable microfluidic-based detectors have been demonstrated, and use optical illumination and detection schemes that are similar in principle to those in bench-top devices [16].
Smartphones have been used with microfluidics for detection and/or optical excitation [14, 17]. One such device is capable of fluorescence-based multiplexed detection of biological pathogens with results available in ~30 minutes [17]. It’s possible to manufacture some optical components (such as lenses) directly onto microfluidic cartridges, to improve performance where the optical path length is necessarily short [16], though this may raise the cost of the disposable component.
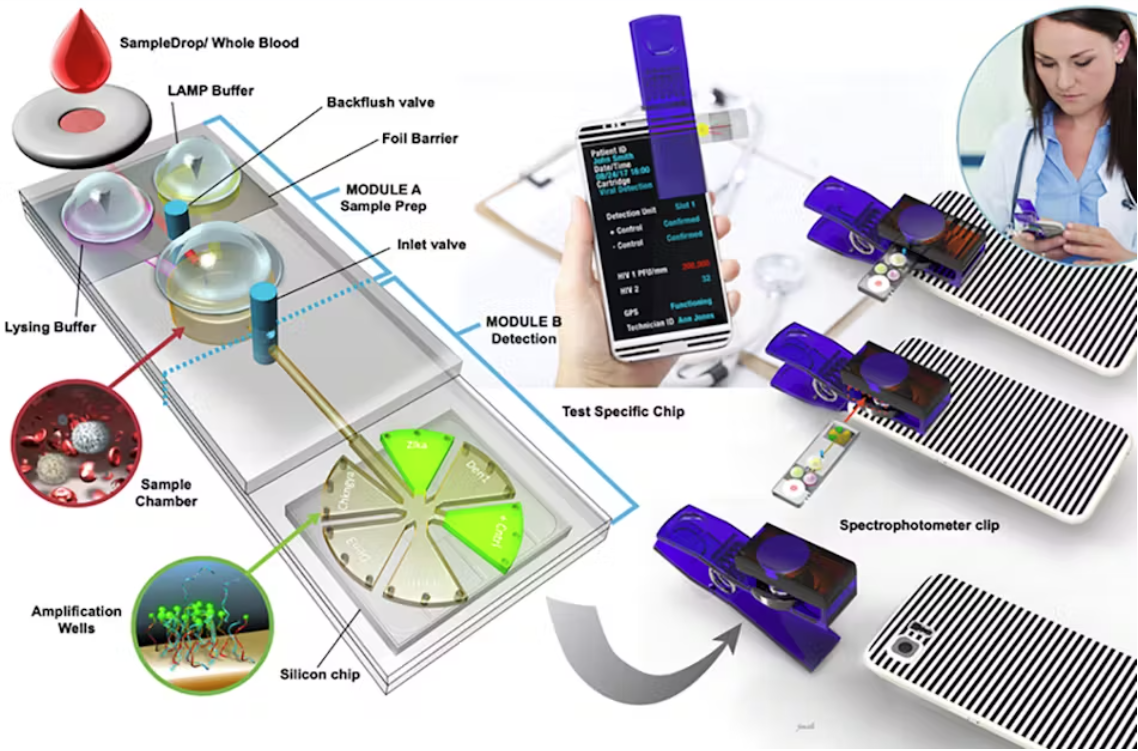
Conclusion
POC diagnostic tools are invaluable for bringing health care to wider groups of patients in varied settings. Optical detection has always been, and continues to be, an integral part of such devices. This blog described some of the ways optical techniques and technologies have been leveraged in these devices in recent years. POC devices have seen much advancement and we can expect to see them become more and more common in the years to come. The optics team at StarFish Medical are always interested in hearing about new applications and devices the use optical technologies for point of care diagnostics. Please contact us to discuss your project.
References
[1] H. Zhu, S. Isikman, O. Mundanyali, A. Greenbaum and A. Ozcan, “Optical Imaging Techniques for Point-of-care Diagnostics,” Lab Chip, vol. 13, no. 1, pp. 51-67, 2013.
[2] S. Sia and L. Kricka, “Microfluidics and point-of-care testing,” Lab Chip, vol. 8, pp. 1982-1983, 2008.
[3] A. St John and C. Price, “Existing and Emerging Technologies for Point-of-Care Testing,” Clin Biochem Rev, vol. 35, no. 3, pp. 155-167, 2014.
[4] M. Pierce, S. Weigum, J. Jaslove, R. Richards-Kortum and T. Tkaczyk, “Optical systems for point-of-care diagnostic instrumentation: analysis of imaging performance and cost,” Ann Biomed Eng, vol. 42, no. 1, pp. 1-19, 2014.
[5] A. Miller, G. Davis, Z. Oden, M. Razavi, A. Fateh, M. Ghazanfari, F. Abdolrahimi, S. Poorazar, F. Sakhaie, R. Olsen, A. Bahrmand, M. Pierce, E. Graviss and R. Richards-Kortum, “Portable, Battery-Operated, Low-Cost, Bright Field and Fluorescence Microscope,” PLoS One, vol. 5, no. 8, p. e11890, 2010.
[6] K. Ghosh, L. Burns, E. Cocker, A. Nimmerjahn, Y. Ziv, A. El Gamal and M. Schnitzer, “Miniaturized integration of a fluorescence microscope,” Nat Methods, vol. 8, pp. 871-878, 2011.
[7] T. Davenport and R. Kalakota, “The potential for artificial intelligence in healthcare,” Future Healthc J, vol. 6, no. 2, pp. 94-98, 2019.
[8] The Verge, “Samsung’s new camera sensor has the tiniest pixels ever,” [Online].
[9] G. Zheng, S. Lee, Y. Antebi, M. Elowitz and C. Yang, “The ePetri dish, an on-chip cell imaging platform based on subpixel perspective sweeping microscopy (SPSM),” PNAS, vol. 108, no. 41, pp. 16889-16894, 2011.
[10] A. Sobieranki, F. Inci, H. Tekin, M. Yuksekkaya, E. Comunello, D. Cobra, A. von Wangenheim and U. Demirci, “Portable lensless wide-field microscopy imaging platform based on digital inline holography and multi-frame pixel super-resolution,” Light Sci Appl, vol. 4, pp. 1-11, 2015.
[11] V. Pamplona, E. Passos, J. Zizka, M. Oliveira, E. Lawson, E. Clue and R. Raskar, “Catra: cataract probe with a lightfield display and a snap-on eyepiece for mobile phones,” Proc. SIGGRAPH, vol. 11, pp. 7-11, 2011.
[12] A. Orth, E. Wilson, J. Thomson and B. Gibson, “A dual-mode mobile phone microscope using the onboard camera flash and ambient light,” Sci Rep, vol. 8, p. 3298, 2018.
[13] B. Dai, Z. Jiao, L. Zheng, H. Bachman, Y. Fu, X. Wan, Y. Zhang, Y. Huang, X. Han, C. Zhao, T. Huang, S. Zhuang and D. Zhang, “Colour compound lenses for a portable fluorescence microscope,” Light Sci Appl, vol. 8, no. 75, pp. 1-13, 2019.
[14] H. Zhu, S. Mavandadi, A. Coskun, O. Yaglidere and A. Ozcan, “Optofluidic fluorescent imaging cytometry on a cell phone,” Anal Chem, vol. 83, no. 17, pp. 6641-6647, 2011.
[15] J. He, D. Wang and S. Fan, “Opto-Microfluidic Immunosensors: From Colorimetric to Plasmonic,” Micromachines, vol. 7, no. 29, pp. 1-18, 2016.
[16] F. Meyers and L. Lee, “Innovations in optical microfluidic technologies for point-of-care diagnostics,” Lab Chip, vol. 8, pp. 2015-2031, 2008.
[17] F. Sun, A. Ganguli, J. Nguyen, R. Brisbin, K. Shanmugam, D. Hirschberg, M. Wheeler, R. Bashir, D. Nash and B. Cunningham, “Smartphone-based multiplex 30-minute nucleic acid test of live virus from nasal swab extract,” Lab Chip, vol. 20, no. 1621-1627, 2020.
Ryan Field is a Senior Optical Systems Engineer at StarFish Medical. Ryan holds a PhD in Physics from the University of Toronto. As a post doctoral fellow, he worked on the development of high-power picosecond infrared laser systems for surgical applications. He has worked in the medical device industry throughout his post-academic career and has worked on a diverse range of optical applications including fluorescence illumination systems, optical assays, diagnostic imaging systems, and ophthalmic laser surgery devices.
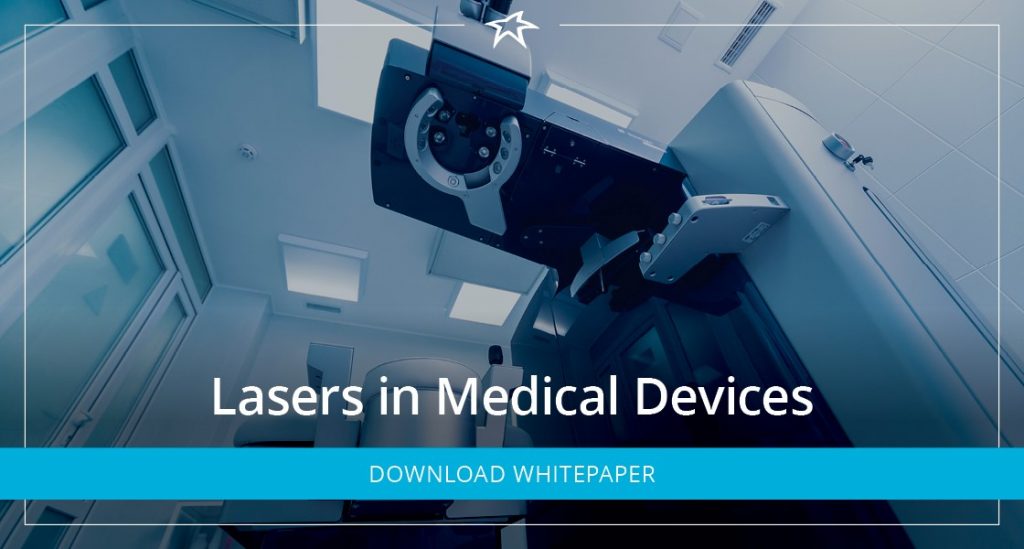